Unraveling the Mystery: Understanding the Dual Nature of Waves and Particles in Quantum Physics
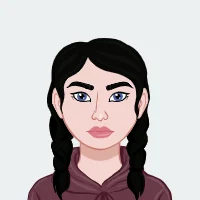
Unraveling the Mystery: Understanding the Dual Nature of Waves and Particles in Quantum Physics" embarks on a captivating exploration of one of the most intriguing phenomena in the quantum realm – the wave-particle duality. This concept, rooted in the fundamental principles of quantum mechanics, challenges classical notions of how particles behave and forces us to reconsider the very fabric of reality. As we navigate through the complex interplay between waves and particles, the journey reveals a tapestry of paradoxes and perplexities that characterize the quantum world. If you need help with your Quantum Physics assignment, delving into the concept of wave-particle duality provides a profound exploration of fundamental principles in quantum mechanics, offering insights into the perplexing nature of quantum phenomena and their implications for our understanding of the universe.
The essence of wave-particle duality lies in the idea that entities like electrons and photons can exhibit both wave-like and particle-like behaviors depending on the experimental conditions. This inherent dual nature prompts profound questions about the nature of matter and energy, inviting us to question our intuitive understanding of the physical world. The iconic double-slit experiment, a cornerstone in quantum physics, serves as a compelling example, illustrating how particles can create interference patterns typically associated with waves when not observed.
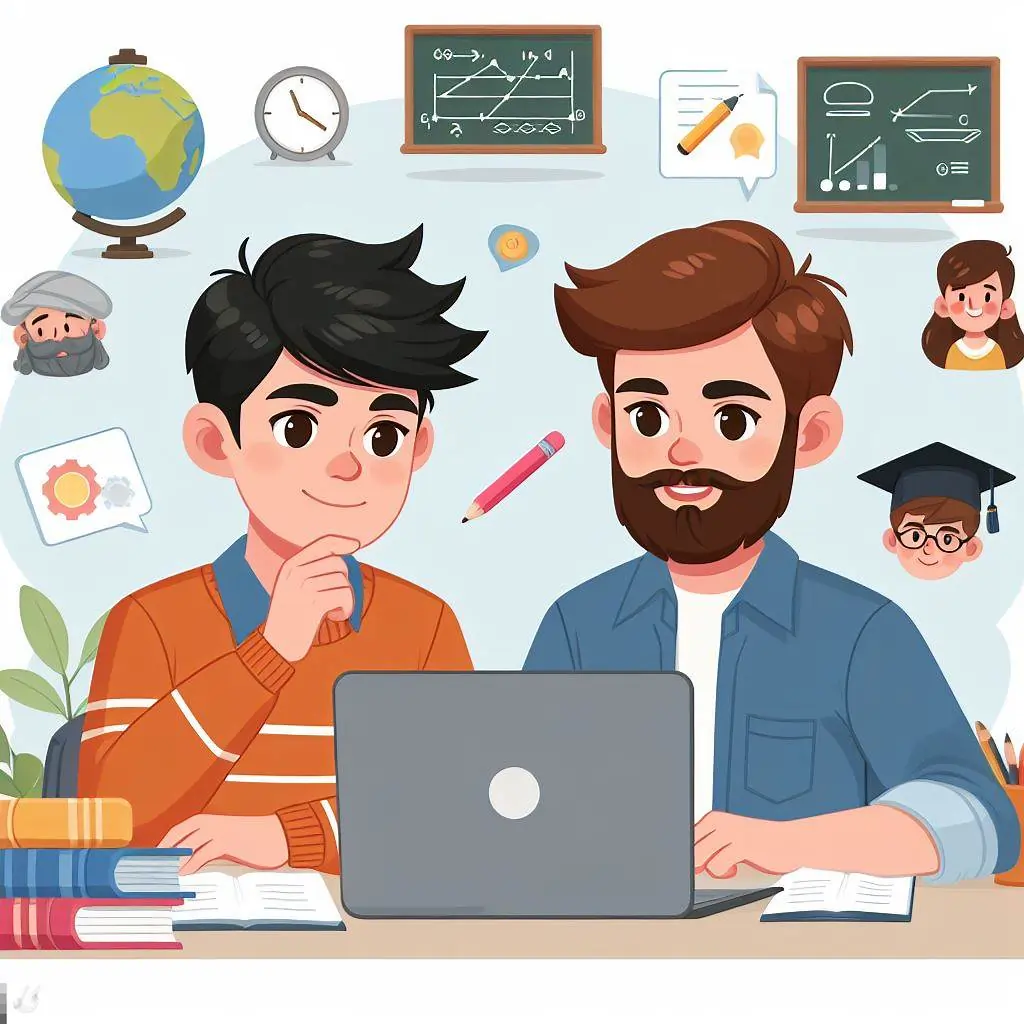
The exploration of wave-particle duality goes beyond mere theoretical musings; it challenges the very foundations of classical physics. The concept implies that particles exist in multiple states simultaneously, a notion that seems counterintuitive within our everyday experiences but aligns seamlessly with the probabilistic nature of quantum mechanics. This unpredictability introduces a level of indeterminacy that distinguishes the quantum world from our macroscopic reality.
Moreover, the implications of wave-particle duality extend far beyond the theoretical realm. The understanding of this phenomenon has paved the way for transformative breakthroughs in technological and scientific arenas. It forms the basis for advancements in quantum computing, quantum cryptography, and the development of cutting-edge technologies that harness the unique features of quantum systems.
"Unraveling the Mystery" is not just an exploration of a scientific concept; it symbolizes the ceaseless quest of physicists to decode the mysteries of the quantum universe. It underscores the persistent fascination and dedication of scientific minds to unravel the complexities of a realm that defies our intuition. The exploration of wave-particle duality stands as a testament to the ongoing dialogue between theory and experimentation, challenging us to expand our understanding of the universe and reshape the boundaries of what we thought possible. In essence, this journey invites us to embrace the paradoxes and uncertainties inherent in the quantum world, fostering a deeper appreciation for the profound mysteries that continue to shape our understanding of the cosmos.
Introduction
In the enthralling exploration of "Unraveling the Mystery: Understanding the Dual Nature of Waves and Particles in Quantum Physics," we embark on a compelling intellectual odyssey that takes us beyond the familiar territories of classical physics into the fascinating and often perplexing realm of quantum mechanics. At the center of our journey lies the enigmatic concept of wave-particle duality, a principle that introduces a profound shift in our understanding of the fundamental nature of matter and energy.
As we venture into the quantum world, our exploration reveals a captivating interplay between waves and particles, exemplified by the inherent dual nature exhibited by subatomic entities like electrons and photons. This dual behavior, wherein these entities can manifest both wave-like and particle-like characteristics depending on experimental conditions, challenges our conventional notions of how physical entities behave. The iconic double-slit experiment, a cornerstone of quantum physics, serves as a poignant illustration, demonstrating how particles can create interference patterns akin to waves when not observed, and yet behave as discrete particles when under observation.
The journey into the complexities of wave-particle duality transcends theoretical discourse; it delves into the very fabric of reality itself. This concept suggests a level of inherent unpredictability and indeterminacy, introducing a paradigm shift that distinguishes the quantum realm from the classical world we observe in our everyday experiences. The exploration prompts profound questions about the nature of reality and calls into question our intuitive understanding of the physical universe.
Moreover, the implications of understanding wave-particle duality extend beyond the theoretical realm, finding practical applications in groundbreaking technologies. The concept forms the bedrock for advancements in quantum computing, a revolutionary field with the potential to transform computation as we know it, and quantum communication, where the unique features of quantum systems are harnessed for secure information transfer.
In essence, this intellectual odyssey invites us to grapple with the paradoxes and mysteries that define the quantum universe. "Unraveling the Mystery" serves as a gateway to a deeper comprehension of the dual nature of waves and particles, encouraging us to question, explore, and appreciate the profound implications that this concept holds for our understanding of the cosmos. As we embark on this journey, we are poised to uncover the secrets that lie at the intersection of theory and experimentation, navigating through the complexities that make quantum physics one of the most fascinating and transformative fields in the scientific landscape.
Historical Perspectives
Delving into the historical perspectives of quantum physics provides a profound insight into the evolution of our understanding of the quantum world. The roots of this scientific revolution can be traced back to the early 20th century, a period characterized by groundbreaking shifts in the conceptual framework of physics.
One of the pivotal moments in the historical narrative is the formulation of quantum theory in the early 1900s. Visionaries like Max Planck, Albert Einstein, Niels Bohr, and Erwin Schrödinger played instrumental roles in unraveling the mysteries of the quantum realm. Planck's groundbreaking work on blackbody radiation introduced the concept of quantization of energy, marking the inception of quantum theory. Einstein's elucidation of the photoelectric effect reinforced the notion of quantized energy and laid the groundwork for the wave-particle duality concept.
Niels Bohr's model of the atom, incorporating quantized electron orbits, further advanced quantum theory. The famous Bohr model successfully explained the spectral lines of hydrogen, a key achievement in understanding atomic structure. However, the limitations of the model became apparent as quantum physics continued to evolve.
The advent of wave mechanics, pioneered by Erwin Schrödinger, and matrix mechanics, developed by Werner Heisenberg, marked a transformative phase in quantum theory during the 1920s. The Schrödinger equation provided a comprehensive framework for describing the behavior of particles as waves, offering an alternative perspective to the particle-centric view of earlier models. Heisenberg's uncertainty principle, unveiled in 1927, emphasized the inherent limitations in simultaneously measuring certain pairs of complementary properties, challenging classical notions of determinism.
The historical narrative also features the development of quantum electrodynamics (QED) and quantum chromodynamics (QCD) in the mid-20th century. Pioneering figures like Richard Feynman and Murray Gell-Mann contributed significantly to these fields, elucidating the quantum behavior of electromagnetic forces and quarks, respectively.
The advent of quantum computing in the latter half of the 20th century and ongoing research into quantum entanglement, superposition, and quantum teleportation showcase the enduring fascination and relevance of quantum physics in contemporary scientific endeavors.
In conclusion, exploring the historical perspectives of quantum physics unveils a narrative of intellectual upheaval, paradigm shifts, and relentless curiosity. The journey through the annals of scientific progress highlights the collaborative efforts of brilliant minds who, over the decades, have reshaped our understanding of the fundamental nature of the universe, leaving an indelible mark on the scientific landscape.
Dual Nature of Light
The dual nature of light is a fundamental concept in physics that emerged from groundbreaking experiments and theoretical developments in the 19th and early 20th centuries. This duality, which encompasses both wave-like and particle-like characteristics of light, challenged conventional understanding and laid the groundwork for the later formulation of quantum mechanics.
The wave nature of light was initially championed by the wave theory of light proposed by Christiaan Huygens in the 17th century. According to this theory, light propagates as a wave through a medium, similar to ripples in water. The wave theory successfully explained phenomena such as diffraction and interference, providing a robust framework for understanding the behavior of light in various situations.
However, in the late 19th century, as physicists explored the mysteries of the photoelectric effect and blackbody radiation, phenomena that couldn't be adequately explained by classical wave theory, the need for a new understanding of light became evident. Albert Einstein's groundbreaking work in 1905, where he introduced the concept of quantized light energy in the form of particles called photons, marked a crucial departure from classical physics. This revolutionary idea, which earned him the Nobel Prize, laid the foundation for the particle nature of light.
The dual nature of light was further illuminated by experiments like the double-slit experiment, conducted first with particles and then with light. When light was shone through two slits, an interference pattern characteristic of waves was observed. This seemingly contradictory behavior demanded a conceptual shift, leading to the understanding that light exhibits both wave-like interference and particle-like quantization, depending on the experimental conditions.
The synthesis of these seemingly opposing aspects culminated in the formulation of wave-particle duality, a central tenet of quantum mechanics. The famous de Broglie hypothesis extended this duality to particles, suggesting that matter, like light, can also exhibit both wave and particle characteristics.
In contemporary physics, the dual nature of light remains a cornerstone of quantum theory. Quantum electrodynamics (QED), a quantum field theory describing the electromagnetic force, incorporates the wave-particle duality of photons. This duality not only explains the behavior of light but also forms the basis for technological advancements, including the development of lasers, quantum optics, and various applications in modern communications and information technology. The dual nature of light, with its rich historical background, stands as a testament to the dynamic and sometimes paradoxical nature of the quantum world
Quantum Waves and Probability
In the realm of quantum mechanics, the concept of quantum waves and probability is fundamental to understanding the behavior of particles at the microscopic level. This notion is deeply rooted in the wave-particle duality, a key principle that emerged in the early 20th century and revolutionized our perception of the quantum world.
Quantum waves are not physical waves in the traditional sense, like water waves or sound waves, but rather mathematical descriptions of the probability distribution of finding a particle in a particular state. These waves are often represented by wavefunctions, which are solutions to the Schrödinger equation—the fundamental equation governing the behavior of quantum systems.
The wavefunction provides information about the amplitude and phase of the quantum wave at each point in space and time. Remarkably, the square of the magnitude of the wavefunction at a given point gives the probability density of finding a particle in that region. This probabilistic interpretation is a departure from classical physics, where particles were traditionally envisioned as having well-defined trajectories.
The famous double-slit experiment exemplifies the role of quantum waves and probability in the behavior of particles. When particles, such as electrons, are sent through two slits, the resulting interference pattern on a screen suggests wave-like behavior. However, when detectors are introduced to determine which slit a particle passes through, the interference pattern disappears. This phenomenon illustrates how the act of measurement affects the probability distribution of the particles and highlights the inherent probabilistic nature of quantum systems.
Furthermore, the uncertainty principle, formulated by Werner Heisenberg, underscores the limitations in simultaneously measuring certain pairs of complementary properties, such as position and momentum. This principle is intimately connected to the spread of the quantum wavefunction and the inherent uncertainties in predicting the precise outcome of measurements.
The probabilistic nature of quantum waves has profound implications for our understanding of reality. It challenges our classical intuition by acknowledging that, at the quantum level, particles do not have definite properties until they are observed. This concept not only distinguishes quantum mechanics from classical mechanics but also forms the basis for quantum superposition, entanglement, and the probabilistic predictions that govern the behavior of particles in the microscopic realm.
In summary, quantum waves and probability are intrinsic to the fabric of quantum mechanics, providing a mathematical framework for describing the behavior of particles in a probabilistic manner. This conceptual shift has revolutionized our understanding of the fundamental nature of reality, ushering in a new era of physics that embraces the uncertainties and intricacies inherent in the quantum world.
Famous Experiments
Several famous experiments in the field of physics have played pivotal roles in shaping our understanding of the fundamental principles that govern the universe. These experiments have not only provided empirical evidence for groundbreaking theories but have also challenged established paradigms, leading to paradigm shifts in scientific thought. Here are some of the most renowned experiments in the history of physics:
- Michelson-Morley Experiment (1887): Conducted by Albert A. Michelson and Edward W. Morley, this experiment aimed to detect the existence of the luminiferous ether, a hypothetical medium thought to carry light waves. The null result of the experiment led to the formulation of Einstein's theory of special relativity.
- Double-Slit Experiment (1801, 1961): Originally performed by Thomas Young, this experiment demonstrated the wave-particle duality of light. Later, the same concept was extended to electrons by Clinton Davisson and Lester Germer. The experiment reveals the interference pattern produced when particles exhibit both wave and particle characteristics.
- Photoelectric Effect (1905): Albert Einstein's explanation of the photoelectric effect, which involves the emission of electrons when light shines on a material, was a cornerstone in the development of quantum theory. Einstein proposed that light consists of discrete packets of energy called photons.
- Stern-Gerlach Experiment (1922): Performed by Otto Stern and Walther Gerlach, this experiment demonstrated the quantization of angular momentum in quantum systems. It provided experimental evidence for the quantized nature of angular momentum and the existence of discrete quantum states.
- Davisson-Germer Experiment (1927): Building on the ideas of the double-slit experiment, Clinton Davisson and Lester Germer observed electron diffraction, providing experimental confirmation of the de Broglie hypothesis that particles, like electrons, exhibit wave-like properties.
- Bell's Theorem and Aspect's Experiment (1964): Physicist John Bell formulated a theorem that challenged classical notions of local realism. Alain Aspect's experiments in the 1980s provided evidence supporting quantum entanglement and demonstrated violations of Bell's inequalities.
- CERN's Large Hadron Collider (LHC): The discovery of the Higgs boson in 2012 at CERN's LHC marked a significant milestone. This experimental achievement confirmed the existence of the Higgs field and contributed to our understanding of the mechanism behind particle mass.
- EPR Paradox (1935): Proposed by Einstein, Podolsky, and Rosen, this thought experiment challenged the completeness of quantum mechanics. While the EPR paradox itself has not been experimentally tested, subsequent experiments like those involving entangled particles have provided insights into the non-local correlations predicted by quantum theory.
These experiments, among others, have not only advanced our understanding of the physical world but have also inspired new theoretical developments, leading to profound shifts in our comprehension of the fundamental laws of nature.
Quantum Superposition
Quantum superposition is a fundamental principle in quantum mechanics that describes the ability of quantum systems to exist in multiple states or configurations simultaneously. This concept challenges our classical intuition, where objects are typically in well-defined states. In the quantum realm, particles such as electrons, photons, and atoms can exist in a superposition of multiple states until a measurement is made, at which point the system "collapses" into one of the possible states.
The superposition principle is mathematically expressed through the quantum wavefunction. A wavefunction describes the probability amplitude of finding a particle in a particular state when measured. When a quantum system is in a superposition of states, its wavefunction is a linear combination of the individual wavefunctions corresponding to each possible state. The probabilities of measuring the system in any one of these states are given by the squared magnitudes of the coefficients in the superposition.
The famous thought experiment known as Schrödinger's Cat illustrates the concept of superposition. In this hypothetical scenario, a cat in a sealed box is in a superposition of being both alive and dead until someone opens the box and makes an observation. This example highlights the peculiar nature of quantum superposition, where macroscopic objects, in theory, could exist in multiple states simultaneously.
The experimental verification of quantum superposition has been demonstrated with various physical systems. One notable example is the interference patterns observed in the double-slit experiment, where particles like electrons exhibit both wave and particle properties. The interference pattern suggests that the particle goes through both slits simultaneously, indicating a superposition of paths.
Quantum computers leverage the principle of superposition to perform parallel computations. Unlike classical bits that exist in states of 0 or 1, quantum bits or qubits can exist in superpositions of both 0 and 1, allowing quantum computers to process information exponentially faster for certain tasks.
However, superposition is fragile and can be easily disrupted by interactions with the environment, leading to a phenomenon known as decoherence. Maintaining and controlling superposition is a significant challenge in the development of quantum technologies.
In summary, quantum superposition is a foundational concept that lies at the heart of quantum mechanics. It reveals the unique and often counterintuitive nature of quantum systems, where particles can exist in multiple states simultaneously, offering both challenges and opportunities for the exploration of quantum phenomena and the development of quantum technologies.
Wave-Particle Duality in Subatomic Particles
Wave-particle duality is a fundamental concept in quantum mechanics that describes the dual nature of subatomic particles, such as electrons and photons. This principle challenges our classical intuition, as it suggests that particles can exhibit both wave-like and particle-like behaviors, depending on the experimental conditions.
The concept of wave-particle duality emerged in the early 20th century as scientists grappled with the behavior of particles at the quantum level. One of the key experiments illustrating this duality is the double-slit experiment. When particles, like electrons or photons, are directed through two slits, they create an interference pattern on a screen, characteristic of waves. However, when the particles are observed or measured, they behave as discrete particles, producing a pattern consistent with individual impacts. This phenomenon highlights the coexistence of wave-like and particle-like properties in subatomic particles.
The de Broglie hypothesis, proposed by Louis de Broglie in 1924, further contributed to the understanding of wave-particle duality. De Broglie suggested that particles, traditionally considered as point-like entities, also exhibit wave-like characteristics. The wavelength of this associated wave is inversely proportional to the particle's momentum, introducing the idea that particles have an inherent wave nature.
The uncertainty principle, formulated by Werner Heisenberg, is another aspect of wave-particle duality. It states that certain pairs of properties, such as position and momentum, cannot be precisely simultaneously known. This inherent uncertainty is a consequence of the wave-like nature of particles, and it fundamentally alters our classical notions of determinism.
Electrons, as subatomic particles, prominently display wave-particle duality. In electron diffraction experiments, electrons exhibit interference patterns similar to those of waves, confirming their wave-like nature. Additionally, electron microscopy relies on the wave nature of electrons to achieve high-resolution imaging.
Photons, which are quanta of light, also exhibit wave-particle duality. The phenomenon of diffraction and interference in light, traditionally understood as wave behavior, is evident in experiments with laser light passing through diffraction gratings. On the other hand, the photoelectric effect, where light ejects electrons from a material, demonstrates the particle-like nature of photons.
The wave-particle duality of subatomic particles challenges our classical intuitions and underscores the need for a quantum description of nature. This duality is a foundational principle in quantum mechanics, influencing our understanding of particle behavior and contributing to the development of quantum technologies, such as quantum computing and quantum communication. It remains a central concept in the ongoing exploration of the intricate and fascinating world of quantum physics.
Quantum Entanglement
Quantum entanglement is a phenomenon in quantum mechanics where two or more particles become correlated in such a way that the state of one particle instantaneously influences the state of another, regardless of the distance between them. This concept, famously referred to by Albert Einstein as "spooky action at a distance," challenges classical intuitions about the independence of distant objects and is a distinctive feature of quantum physics.
The entangled particles, often referred to as entangled pairs, can be, for example, photons, electrons, or other quantum particles. When particles become entangled, their individual quantum states become interdependent. The interesting aspect is that, even if these entangled particles are separated by vast distances, a change in the state of one particle will immediately affect the state of the other, a phenomenon often described as "non-local."
The entanglement of particles is typically created through processes such as spontaneous parametric down-conversion or the decay of certain types of particles. Once entangled, the quantum state of each particle is no longer independent but is intrinsically linked to the state of its entangled partner.
One of the most well-known aspects of quantum entanglement is the violation of Bell inequalities. Physicist John Bell proposed these inequalities as a way to test the limits of classical explanations for the correlations observed in entangled particle experiments. Numerous experiments, starting with the work of Alain Aspect in the 1980s, have consistently demonstrated violations of Bell inequalities, providing strong evidence for the unique and non-classical nature of entanglement.
Entanglement has practical implications in the development of quantum technologies. Quantum entanglement forms the basis for quantum teleportation, a process where the quantum state of a particle is transmitted from one entangled particle to another, without a physical transfer of the particle itself. This has potential applications in quantum communication and quantum computing.
Moreover, quantum entanglement plays a role in quantum key distribution (QKD), a method for secure communication. Changes in the entangled states of particles can be used to detect eavesdropping attempts, providing a secure means of transmitting cryptographic keys.
Despite its fascinating and well-established experimental evidence, the underlying mechanisms and the nature of the connection between entangled particles remain topics of ongoing research and philosophical debate within the field of quantum physics. Quantum entanglement stands as one of the most intriguing and counterintuitive features of the quantum world, challenging our classical notions of separability and opening new avenues for exploration in both fundamental physics and practical applications.
Applications and Technological Impacts
The principles of quantum mechanics, including concepts like superposition, entanglement, and wave-particle duality, have led to a variety of applications and technological impacts. These quantum technologies harness the unique properties of quantum systems to perform tasks that are not achievable with classical technologies. Here are some notable applications and technological impacts of quantum mechanics:
Quantum Computing:
- Impact: Quantum computers have the potential to solve certain complex problems exponentially faster than classical computers. They use quantum bits or qubits, which can exist in superpositions of 0 and 1, allowing for parallel processing of information.
- Applications: Quantum computing can revolutionize fields such as cryptography, optimization problems, and drug discovery by efficiently solving problems that are currently intractable for classical computers.
Quantum Communication:
- Impact: Quantum communication ensures secure transmission of information by utilizing the principles of quantum entanglement. It offers a level of security that is theoretically unbreakable due to the principles of quantum key distribution (QKD).
- Applications: Quantum communication can be used for secure communication channels, quantum key distribution for cryptography, and the development of quantum networks.
Quantum Sensing and Metrology:
- Impact: Quantum sensors leverage quantum properties to achieve unprecedented levels of precision in measuring physical quantities. This can lead to advancements in fields such as navigation, imaging, and detection.
- Applications: Quantum sensors can be used in fields like healthcare for precise medical imaging, environmental monitoring, and navigation systems.
Quantum Cryptography:
- Impact: Quantum cryptography uses quantum properties to create secure communication channels, preventing eavesdropping and ensuring the privacy of transmitted information.
- Applications: Quantum key distribution (QKD) is a practical application of quantum cryptography that is being explored for secure communication in various industries, including finance and government.
Quantum Imaging:
- Impact: Quantum imaging techniques exploit the quantum properties of particles of light (photons) to achieve high-resolution imaging beyond the classical limit.
- Applications: Quantum imaging can be applied in fields such as medical imaging, microscopy, and remote sensing, offering improved resolution and sensitivity.
Quantum Simulation:
- Impact: Quantum simulators model the behavior of quantum systems, providing insights into complex physical phenomena that are challenging to study using classical computers.
- Applications: Quantum simulation can be employed in materials science, chemistry, and physics to understand and simulate quantum systems, leading to the discovery of new materials and drugs.
Quantum Metrology:
- Impact: Quantum metrology utilizes quantum states to improve the precision of measurements, surpassing the limits set by classical measurement techniques.
- Applications: Quantum metrology can enhance the accuracy of measurements in fields such as timekeeping, gravitational wave detection, and magnetic field sensing.
While many of these technologies are still in the early stages of development, they hold great promise for transforming various industries and solving problems that were once considered intractable using classical approaches. The ongoing progress in the field of quantum technology continues to open new frontiers in science, computation, and communication.
Future Frontiers
The future of quantum mechanics and its applications holds exciting possibilities, promising to reshape various aspects of technology, science, and our understanding of the universe. Here are some potential future frontiers in the field:
Quantum Computing Advancements:
- Quantum Supremacy: Continued efforts in building larger and more stable quantum computers aim to achieve quantum supremacy, where these machines can perform tasks surpassing the capabilities of classical computers.
- Quantum Error Correction: Developing robust quantum error correction techniques is crucial for scaling up quantum computers and making them more fault-tolerant.
Quantum Communication Networks:
- Global Quantum Internet: The establishment of a global quantum internet could enable secure communication worldwide using quantum key distribution (QKD). Research is underway to develop quantum repeaters for long-distance quantum communication.
Quantum Sensing and Imaging:
- Medical Applications: Quantum sensors and imaging technologies may find applications in medical diagnostics, enabling more precise and non-invasive imaging techniques.
- Environmental Monitoring: Quantum sensors could contribute to enhanced environmental monitoring, providing high-precision measurements for climate research and pollution detection.
Quantum Materials and Technologies:
- Quantum Materials Design: Advancements in the design and synthesis of quantum materials may lead to the development of novel materials with unique properties for applications in electronics, energy storage, and beyond.
- Topological Quantum Computing: Exploring topological quantum states of matter could pave the way for the realization of more stable and fault-tolerant qubits for quantum computing.
Quantum Machine Learning:
- Quantum Machine Learning Algorithms: The integration of quantum computing with machine learning could lead to the development of more efficient algorithms for tasks such as optimization, pattern recognition, and data analysis.
Quantum Biology:
- Understanding Biological Processes: Investigating the potential role of quantum effects in biological processes could provide insights into the mechanisms of photosynthesis, enzymatic reactions, and other fundamental biological phenomena.
Quantum-enhanced Technologies:
- Quantum-enhanced Metrology: Further advancements in quantum metrology may lead to highly precise measurement devices, impacting fields such as gravitational wave detection and navigation systems.
- Quantum-enhanced Imaging: Quantum imaging techniques could be refined for applications in medical imaging, security, and remote sensing.
Quantum AI and Optimization:
- Quantum Machine Learning Applications: Quantum algorithms may be harnessed to solve optimization problems relevant to fields such as logistics, finance, and resource allocation.
- Hybrid Quantum-Classical Approaches: Integrating quantum and classical computing for hybrid approaches to optimization problems could improve the efficiency of solving complex computational tasks.
Fundamental Quantum Physics:
- Testing the Foundations: Ongoing experiments aim to test the foundations of quantum mechanics, exploring questions related to the nature of entanglement, non-locality, and the fundamental limits of quantum systems.
Quantum Computing in Chemistry and Material Science:
- Simulation of Complex Molecules: Quantum computers may be utilized to simulate the behavior of complex molecules, revolutionizing drug discovery and materials science.
- Catalyzing Discoveries: Quantum computing holds the potential to accelerate the discovery of new materials with desirable properties for various applications.
As research and development in quantum mechanics continue, these future frontiers represent only a glimpse of the transformative potential of quantum technologies. The interdisciplinary nature of quantum science is likely to lead to unexpected breakthroughs, opening new avenues for exploration and innovation. The coming decades are poised to witness the integration of quantum technologies into everyday life, revolutionizing how we approach computation, communication, and problem-solving
Conclusion
In conclusion, the landscape of quantum mechanics continues to unfold, revealing a myriad of possibilities that stretch across the realms of technology, science, and our fundamental understanding of the universe. The principles of quantum mechanics, once considered abstract and esoteric, are now propelling us into a future where the potential for innovation and discovery seems boundless.
From the groundbreaking concepts of superposition and entanglement to the development of quantum technologies like computing, communication, and sensing, the impact of quantum mechanics is profound and far-reaching. Quantum computing, in particular, stands on the cusp of a revolution, promising to solve complex problems that were once deemed insurmountable.
As we peer into the future, the realization of a global quantum internet, secure communication through quantum key distribution, and the emergence of quantum-enhanced technologies in sensing, imaging, and materials science beckon. The interdisciplinary nature of quantum science not only challenges classical paradigms but also invites collaborations that transcend traditional boundaries, fostering a convergence of physics, computer science, materials science, and biology.
The journey into the quantum frontier is marked by ongoing exploration, experimentation, and theoretical innovation. Fundamental questions about the nature of reality and the intricacies of quantum phenomena continue to captivate scientists and spark curiosity. As quantum technologies evolve, so too will our ability to unravel the mysteries of the quantum realm, paving the way for unprecedented advancements and paradigm shifts in various fields.
The future of quantum mechanics holds the promise of transformative breakthroughs, ushering in an era where quantum technologies become integral components of our technological landscape. From quantum-enabled advancements in healthcare and environmental monitoring to the revolutionary capabilities of quantum computing, the quantum revolution is poised to leave an indelible mark on the scientific and technological tapestry of the 21st century.
In this ever-expanding quantum frontier, the pursuit of knowledge and the quest for technological innovation converge, promising a future where the once-unimaginable becomes the new reality. As we navigate this quantum landscape, the journey continues, fueled by the curiosity to unravel the mysteries of the quantum world and harness its potential for the betterment of society and our understanding of the cosmos.